Breakthroughs in battery science by Drexel engineers are paving the way for new tech that can solve big energy problems. In this package of stories from the College of Engineering, hear about the worldwide hunt for a killer app for MXenes…learn about a new method of making nanomaterials that could be a source safe hydrogen energy…follow one researcher’s path toward commercializing a drone battery…and discover how researchers are keeping electric vehicle batteries charged and cooled.
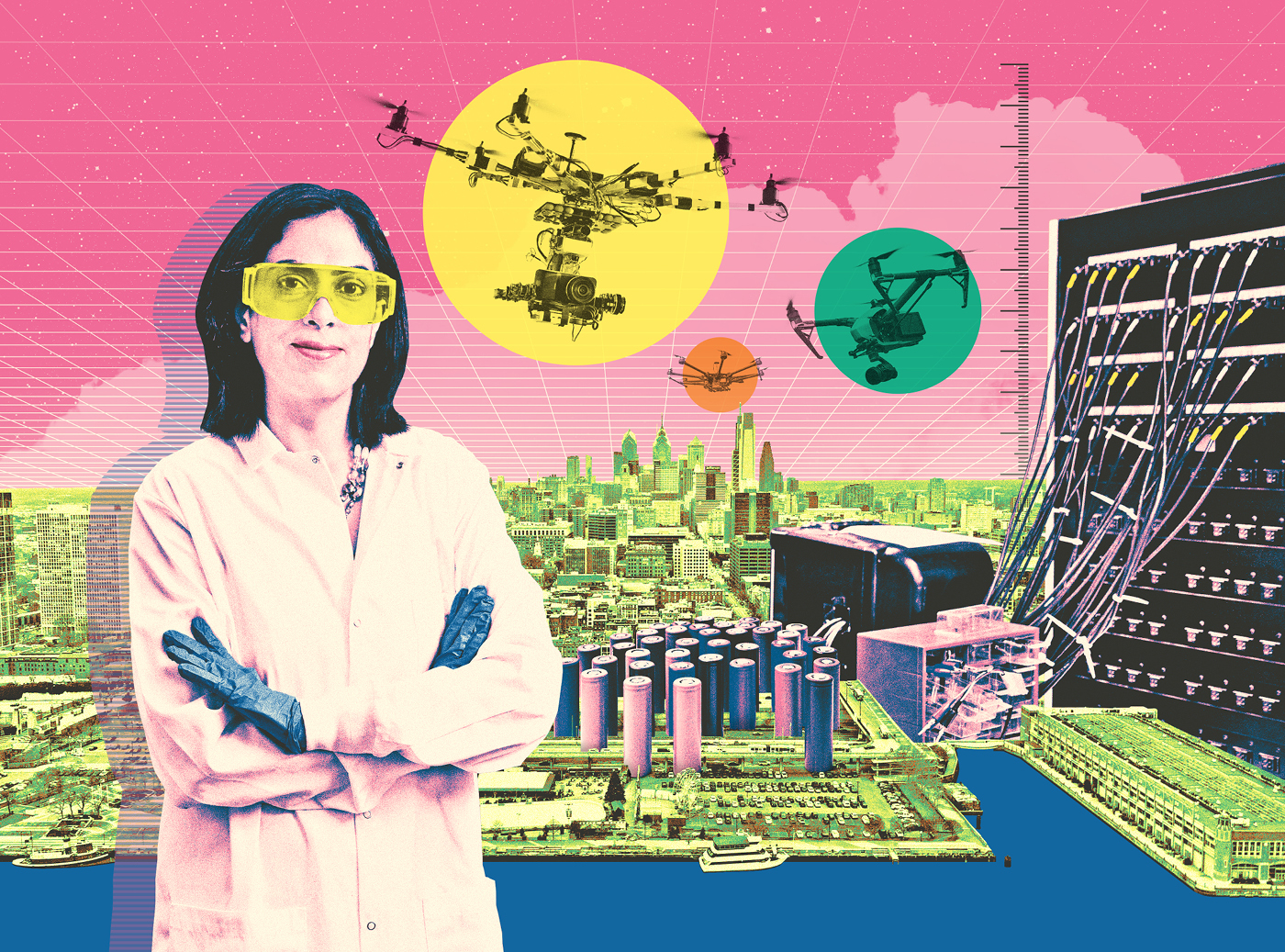
The_Path_to_Lift-off
_by Christina Hernandez Sherwood
The discovery of a stable sulfur-based battery could lead to a lightweight, longstanding battery for aerial drones.
In the mid-1980s, Sony executives were under pressure.
The corporation was racing to develop a high-density, rechargeable battery that could power its electronics again and again. But Union Carbide had just pulled out of their R&D joint venture and a rival Japanese firm already had a battery prototype, recounts Charles J. Murray in the book “Long Hard Road: The Lithium-Ion Battery and the Electric Car.” In 1987, the head of Sony’s battery development subsidiary, Keizaburo Tozawa, shifted to high gear, assigning six separate research groups to focus solely on this mission. Two and a half years later, Sony announced its “dream battery,” a rechargeable lithium-ion battery that was safer and more powerful than its competitors.
Fast-forward three decades: These revolutionary lithium-ion batteries, first used in Sony camcorders, now power everything from the smartphones in our pockets to the electric cars in our driveways. They have seen vast improvements over the past 30 years — most notably in the amount of energy they can store — and are still considered the gold standard rechargeable battery.
But every technology has its limits, and the lithium-ion battery is bumping up against its limits in the electric vehicle market. For a lithium-ion battery to store more energy — to run longer between charges — it must weigh more. While that increased weight isn’t much of a problem in small electronics, it is an issue in vehicles. The lithium-ion battery powering a Tesla, for instance, weighs more than 1,000 pounds and accounts for as much as half of the car’s total cost.
“That’s not sustainable,” says Vibha Kalra, who is the George B. Francis Chair Professor and director of the PhD Program in Drexel’s Department of Chemical and Biological Engineering within the College of Engineering. “When you try to translate the same technology into larger vehicles, it’s not going to be sustainable with respect to both weight and cost. A pickup truck would require at least twice the battery storage of a sedan. A commercial truck could require almost tenfold.”
Kalra is working to bridge this gap. In her decade-long journey to create and commercialize a new generation of rechargeable batteries, Kalra has performed hundreds of experiments, interviewed dozens of industry experts, received close to $7 million in grants and funding, logged countless hours working with tech commercialization experts in Drexel’s Office of Research & Innovation, and secured three patents, with 11 more pending.
Though the work isn’t over, the path of Kalra’s research discovery from lab bench to a top commercial prospect exemplifies the power of academic entrepreneurship in supercharging faculty careers and expanding the University’s research enterprise.
“It gives a good picture of what it’s like to take a really early-stage, interesting technology to the point where it’s ready to make its way into a company,” says Robert McGrath, senior associate vice provost for intellectual property and agreements in Drexel Applied Innovation, the technology transfer division of the Office of Research & Innovation. “Getting to market [is hopefully] not far behind.”
The discovery
Chemical engineers have long been intrigued by the potential of combining lithium with sulfur to make a new kind of battery. The opportunity is enticing: Lithium-sulfur batteries have the potential to store more than five times the energy of their lithium-ion counterparts. As a widely abundant material, sulfur is super cheap. It’s also benign, unlike some heavy metals and materials that face environmental and humanitarian issues as well as looming shortages.
All of this promises an affordable, abundant, lighter, greener battery — with spectacular performance in everyday electronics. Imagine running your laptop for three times as long, or getting the same run time from a laptop that weighed a third less. An electric car with a lithium-sulfur battery would have a range of 1,500 miles, versus 500 miles for a lithium-ion battery of the same weight.
But Kalra and other researchers were stumped by a critical obstacle to using sulfur. Both lithium-ion and lithium-sulfur batteries contain a liquid, called electrolyte, that transports lithium ions from one side of the battery to the other, enabling its charge and discharge cycle. Most experimenters studying lithium-sulfur batteries have favored ether electrolyte because it plays well with sulfur. However, it has a low boiling point, making it too dangerous for practical use.
Kalra and her PhD student decided to ditch ether electrolyte in favor of carbonate electrolyte, which has been used in lithium-ion batteries for 30 years.
“It’s the path of least resistance,” Kalra explains. “Rather than pushing for the industry adoption of a new electrolyte, our goal was to make a cathode that could work in the pre-existing Li-ion electrolyte system.”
Of course, it isn’t as easy as swapping one electrolyte for the other. When Kalra first put carbonate electrolyte in an experimental lithium-sulfur battery, the battery shut down after its first cycle. To counteract the adverse reaction between sulfur and carbonate electrolyte, the team tried to isolate sulfur from the carbonate by enmeshing it in a carbon nanofiber substrate using a vapor deposition technique.
They didn’t get the result they expected. But as they explain in their paper published in Communications Chemistry, what they got instead was a game changer: a rare form of crystalized sulfur, called monoclinic gamma sulfur, that is stable at room temperature. Long-term stability of this form of sulfur had never been achieved in a lab before.
“As we began the test [of the cathode], it started running beautifully — something we did not expect,” Kalra recalls. “In fact, we tested it over and over again — 50–100 times — to ensure we were really seeing what we thought we were seeing.”
When the team put their stable sulfur into a battery, they were able to cycle it 4,000 times, or the equivalent of 10 years of use, without losing performance. In contrast, a typical lithium-ion battery can cycle 2,000 times and has only a third of the energy density.
With her discovery confirmed, Kalra called Drexel Applied Innovation.
First steps
Drexel Applied Innovation is the university office responsible for helping faculty, postdocs and graduate students expand the impact of their research results through technology transfer. Kalra had been on the office’s radar since she first met McGrath in 2011. At that first meeting, Kalra didn’t have a discovery yet, but she told McGrath what he could expect from her lab.
“I thought it was relevant,” McGrath says, “but as with most of the things coming out of a university lab, it was probably ahead of its time. It usually takes a good five, 10 years — and in some cases 15 — for industry to catch up to where universities happen to be going.”
Kalra continued meeting with the team over the next several years, keeping staff updated on the progress of her research. Elizabeth Poppert, a licensing manager for Drexel Applied Innovation, says Kalra was initially skeptical that industry would be interested in her lithium-sulfur work.
“But after talking to some of our strategic partners, and seeing their excitement, it made her understand that maybe technologies that are still a way away could attract industry,” Poppert says.
The buzz from industry partners convinced the University’s Office of Research & Innovations to provide her with $100,000 in early-stage support.
Sensing the commercial potential for her work, Kalra also reached out frequently to the University’s media relations team to publicize her findings beyond the academic community. A member of that team, Britt Faulstick, helped to translate her findings for the general public.
In 2017, one of his articles happened to catch the eye of a company. They reached out to Kalra about a licensing agreement, and ultimately decided to sponsor her research up to $1 million.
“It was ahead of its time. It usually takes a good five, 10 years — and in some cases 15 — for industry to catch up to where universities happen to be going.”
Robert McGrath, senior associate vice provost for intellectual property and agreements in Drexel Applied Innovation
“This is a great example of what we’re hoping to achieve through the media relations process,” says Faulstick, executive director of news and media relations in the University Marketing & Communications division. “Not only did promoting her work open exciting opportunities for Dr. Kalra, but it also raised awareness of the quality of research taking place at Drexel.”
The partnership enabled Kalra to access equipment that would grow her experimental batteries from tiny coin cells to larger pouch cells, a key step on the path to commercialization. She ramped up her research, performing dozens of fundamental studies — from electrochemistry analysis to postmortem spectroscopy — to confirm and understand her discovery.
At the same time, the team at Drexel Applied Innovation kicked their efforts into high gear. “When the company came knocking on her door,” McGrath says, “that was validation.”
Kalra had already filed a provisional application by then, but it was time to ramp up the process.
The patents
When a faculty member contacts Drexel Applied Innovation with a potential discovery — as more than 100 typically do each year — the team uses two crucial questions to decide whether to take on the project: Is the discovery new, and therefore, patentable? And would industry be interested?
The Drexel Applied Innovation team set to work answering the first question for Kalra’s technology. With the help of patent attorneys, and input from Kalra, the team searched for potential competitors. After submitting each of Kalra’s three patent applications, the team braced for an almost-certain initial rejection. Then the team, including Kalra, revised each application to address the patent office’s objections.
“It’s a significant amount of time,” McGrath says. “I don’t want to say it’s not for the faint of heart, but it is when you have classes to teach and research to do and professional development and faculty service. The folks who do it are committed to seeing their technologies go somewhere.”
Kalra was eventually issued all three patents in 2021, each taking two to four years from initial application to final approval. McGrath called this timeframe “actually pretty reasonable” considering he’s seen others that have taken more than a decade.
Fortunately, Drexel Applied Innovation’s second question — did the discovery have industry appeal? — had already been answered by Kalra’s new corporate partner. “It wasn’t lip service,” McGrath says. “It spoke volumes about [Kalra] and the expertise of her laboratory that the company’s investment, at the time, may have been one of the largest single-year industry-sponsored research projects Drexel had done.”
Eventually, though, the company decided to move its business away from batteries, and the licensing agreement came to an amicable end.
Entrepreneur-in-training
Nonetheless, Kalra had proven her technology had industry appeal. The next step was to home in on the ideal industry niche for her lithium-sulfur batteries. At that point, electric vehicles were still the frontrunner.
Kalra received a National Science Foundation Partnership for Innovation grant, which helps academics move their technologies toward commercialization. A key aspect of the program is participation in National Science Foundation I-Corps, a customer discovery entrepreneurial training program. NSF I-Corps is designed to help faculty determine who outside academia would care — and how much — about their innovation, says Shintaro Kaido, vice provost for innovation and executive director of Drexel Applied Innovation. (Drexel became a member of the $15 million NSF I-Corps Hub Northeast Region in 2022.)
“[Kalra’s] innovation with lithium-sulfur has interesting properties that current lithium-ion batteries don’t,” Kaido says. “How is the technology’s unique value proposition poised to solve problems that current lithium battery technologies can’t address?”
Kalra was eager to interact with industry again, which is critical to the technology transfer process, says Poppert. “We can do some initial interrogations to determine if there’s interest,” she says, “but if we can’t engage the faculty to participate, then it’s not going to go anywhere.”
Kalra didn’t go through I-Corps alone. Her first step was to form a team that was meant to simulate an early-stage startup. Kalra was the technical lead. Her graduate student was the entrepreneurial lead. Poppert and Mohammad Balapour, who is entrepreneur in residence at Drexel Applied Innovation, signed on as mentors.
The team tapped into their professional networks, and even searched LinkedIn, for contacts at dozens of companies across the entire battery ecosystem, ranging from material suppliers to battery manufacturers to end-application firms. All told, the team conducted 106 interviews — giving them deep knowledge of the complex battery landscape.
“It provided a depth of understanding of how that industry works and the challenges of bringing a new innovation to that industry,” Poppert says. “You want to innovate to create a solution that people actually care about.”
While it was clear the industry cared about electric vehicles, the team learned that the market is rigid, with many partnerships already locked in. Although the electric vehicle market is still part of the team’s long-term strategy, their first target industry shifted from land to sky.
“The results of I-Corps were phenomenal,” Kalra says. “We discovered this niche application of aerospace and aviation where such a product could be targeted as a test case.”
Unmanned vehicles like drones face less stringent safety standards than electric vehicles, making the smaller niche market an easier initial target for a new technology like Kalra’s. Plus, battery weight becomes more important in an object that’s meant to leave the ground, so the lightness of her lithium-sulfur batteries had obvious appeal to aerospace.
“It’s not the massive market initially, but we can do some things now to get into the smaller market,” McGrath says. “Then when the [electric vehicle] industry comes around, we’re going to be in a good position to capitalize on that.”
Launching a startup?
While the team was going through the I-Corps process, Drexel’s internal news team published another story about her research — and once again, it attracted attention from a would-be partner.
The team was approached by an entrepreneur who is now in good faith discussions with Drexel as he develops strategic partners and works to form a company. It could still be months before a deal is signed, if at all.
“At the end of the day, we want to make sure the deal we do with the partner is the right partner for [Kalra], the right partner for Drexel,” McGrath says, “and it puts the technology in the best position to be successful and come to market.” (If a startup is formed, it could take advantage of the University’s new Innovation Fund, which will invest $150,000 in up to four Drexel-born startups each year.)
As possible commercialization moves forward on the business side, Kalra’s science is also pushing toward that goal. Her mission now is to ensure her technology can be productized — that is, can it be repeated at scale in a way that is both cost efficient and meets performance requirements?
“It’s one thing to do [this work] on a bench,” Poppert says. “It’s much different to be able to do millions or billions of products and know it’s cost effective and you’ve got the supply in order and the production capability to meet the demand. So much more goes into the business aspect of [tech transfer].”
So Kalra is back in the lab performing extensive experiments on each of the many individual components of her lithium-sulfur battery, and on the battery as a whole. She needs to scale up each component as she works toward her first big milestone: building a prototype, which brings its own set of material and assembly challenges.
But such is the excitement and uncertainty of bringing scientific discoveries to market. Despite years of work and much progress, it’s still an open question whether Kalra’s technology will take off and one day power drones in the sky.
“It could fill a very interesting spot in that ever-changing landscape of stored energy innovation,” Kaido reflects. “We’re really excited, but the truth is we don’t know.
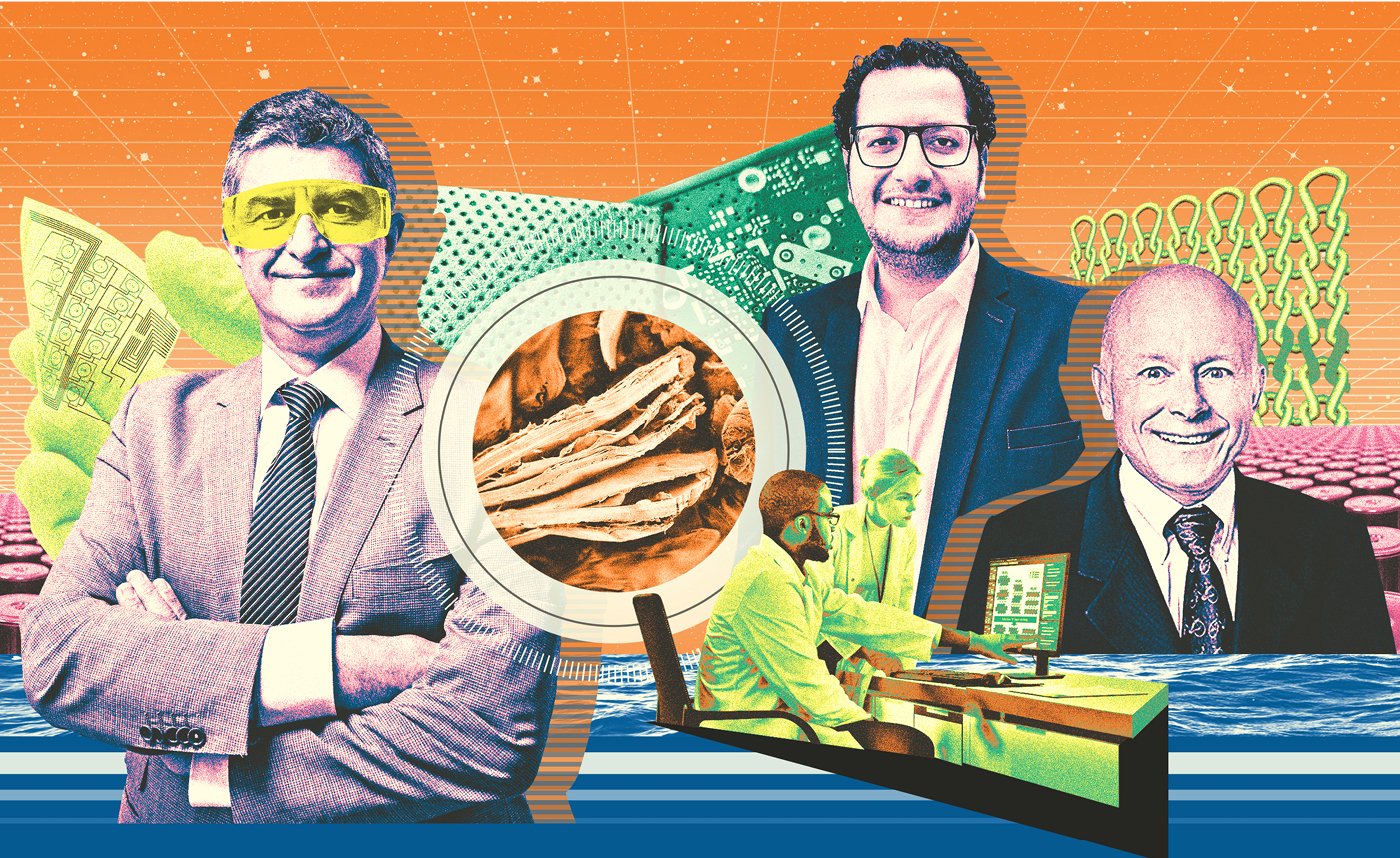
The_Great_Conductor
_by Ben Seal
A global network of scientists has coalesced around the desire to solve big energy problems with tiny nanomaterials discovered at Drexel
Part I: The Discovery
The story of the nanomaterial that could change the world begins with failure.
In 2010, in a lab inside Drexel’s Department of Materials Science and Engineering, first-year PhD student Michael Naguib was trying to squeeze lithium ions into the atoms-thin silicon layers of a material called a MAX phase, in hopes of creating a new battery material for the U.S. Department of Energy. He wanted to take advantage of the potential of MAX phases — carbides and nitrides that merge the properties of metal and ceramic materials — to create a battery anode that would be more robust than state-of-the-art graphite.
Under the co-supervision of Michel Barsoum, who first revealed the properties of MAX phases in the 1990s, and Yury Gogotsi, who was studying the use of carbons produced by selective removal of metals from carbides — including MAX phases — for electrochemical energy storage, Naguib began experimenting.
Thinking particle size could be standing in the way, he first subjected MAX phases to a high-energy collision procedure to break them down to the nanoscale, but he found no improvement in performance. He attempted next to create vacancies in the MAX phases, removing the thin silicon or metal layer in the middle of their sandwiched structure to offer lithium entry. Yet each attempt failed to demonstrate the necessary electrochemical activity.
Finally, he tried etching MAX phases in hydrofluoric acid, hoping to both reduce the particle size and extract the metal layers. The results opened a new era in materials science.
At first, what he got looked as if it might be graphene, an atom-thick form of carbon that had just been awarded the Nobel Prize in physics. But it turned out to be something far more exciting. Though they had failed in their attempt to get lithium into MAX phases, the researchers had discovered something special: a two-dimensional sheet of transition metal carbide that further experimentation developed into an entire family of highly conductive, mechanically robust, water-dispersible and tunable materials that are now being explored by researchers across the world for their potential to change how science approaches energy storage, technology, medicine, environmental remediation and more. The trio named it MXenes, a splice of “MAX phases” (without the A metal, which was dissolved in acid) and “graphene.”
“There’s always a role of serendipity in scientific discoveries,” says Gogotsi, the Distinguished University and Charles T. and Ruth M. Bach Professor in the College of Engineering and director of the A.J. Drexel Nanomaterials Institute. “But, as Pasteur said, ‘Fortune favors the prepared mind.’”
Next, they set out to learn as much as they could. Every graduate student around suddenly took an interest, looking to join the team with Gogotsi and Barsoum, Distinguished Professor in the Department of Materials Science and Engineering.
“We called it, between us, the MXene vortex,” Gogotsi says. “Anybody who came close to it would be sucked in. People quickly realized it was very novel and very important. They saw the sparkle in our eyes when we talked about it.”
In the decade-plus since the discovery, the vortex has expanded to attract the interest of researchers at hundreds of institutions in more than 90 countries on six continents. Through research collaborations, international conventions, alumni networks and no small amount of enthusiasm orchestrated by Gogotsi, researchers around the world have coalesced around an international effort to uncover the extraordinary range of possibilities presented by MXenes’ unique traits.
“We have the building blocks,” Gogotsi says. “Now, we need to learn how to use them to build new technologies.”
Part II: A Teenager in Love
Gogotsi grew up in Kyiv, Ukraine, when it was still part of the Soviet Union. His father, George, a mechanical engineer, encouraged him to take apart telephones and other common devices to understand how the world around him worked. His love of science, though, centered on chemistry. He missed the opportunity to get a textbook for his eighth-grade class, but it didn’t stop him from learning everything he could.
“I was a natural, imbibing all the knowledge,” Gogotsi says. “I didn’t need a textbook because everything was clear to me.”
From the moment he discovered chemistry, he knew his life would be in the sciences. As he watched the instructor of his local chemistry club turn a copper coin into silver by coating it with mercury and create chaos by throwing sodium into water, he experienced something like love at first sight.
After his father introduced him to metallurgy, where he could merge his devotion to chemistry and his fascination with high temperatures, he went to Kyiv Polytechnic Institute, where he earned a master’s degree in metallurgy and a PhD in physical chemistry, setting the stage for a career studying materials chemistry and ceramics. In the process, he learned a lesson that has stuck with him ever since.
At the time, not long after the global oil crisis of the 1970s, researchers around the world were searching for ways to replace the metal in car engines with more efficient, lighter and more heat-resistant ceramics. In an era of hulking gas guzzlers, their work was viewed as critical to society’s path forward. And they achieved their goal, building prototypes in the 1980s that led to the world’s first car with a ceramic turbocharger and the testing of full ceramic engines.
But the ceramic engine never took off. It was an engineering marvel that couldn’t overcome challenges with manufacturing, cost and mass production. Oil prices stabilized, metallic engines improved and, ultimately, there was no need for ceramics in engines.
“Not everything we make that’s good from an engineering standpoint becomes industrial,” Gogotsi says.
More than three decades later, he’s working to ensure that MXenes don’t suffer the same fate.
As soon as Mikhail Gorbachev opened the doors to the Soviet Union, Gogotsi eagerly left to continue his studies on a Humboldt Research Fellowship at the Karlsruhe Institute of Technology in Germany. Before joining Drexel in 2000, he moved between institutions in Japan, Norway, Germany and Chicago, learning more at each step about the most advanced materials in the world. His search led him to carbon nanomaterials, which he focused on for more than 20 years before the discovery of MXenes. That was a moment that changed his life.
“It’s very difficult to imagine the excitement of seeing something no one else in the world has ever seen,” Gogotsi says. “It’s an unbelievable feeling.”
He compares scientific discovery to the feeling some people chase by cliff-jumping or climbing mountains. When he sits down to write a paper describing a new finding, he puts everything else aside to work through the night until he’s emptied all of his ideas onto the page. Only then can he take a deep breath and turn his attention elsewhere.
“It’s the novelty of it,” Gogotsi says. “And the more novel it is, the more unusual, the more exciting.”
In MXenes, he had found with Barsoum and Naguib something entirely new.
Part III: The Vortex Expands
If it was immediately clear to the team at Drexel that this was a major discovery, the scientific community took slightly longer to realize it. Their paper detailing the findings was rejected from two journals that doubted whether MXenes could outperform other two-dimensional materials. It was only accepted by a third journal, Advanced Materials, after a second review on appeal. Years later, the journal’s editor approached Gogotsi at a conference with a bottle of champagne, celebrating what has since become one of the most cited papers in the field. It earned a 10th anniversary retrospective in Advanced Materials in 2021.
In that time, the MXene family has expanded to include dozens of different materials, depending on which ingredients are used in the process. For Gogotsi, this is both a blessing and a challenge. The variation from one MXene to the next is essential to the materials’ tunability — their capacity to be everything to everyone, depending on the task at hand. MXenes are “a kind of black box,” Chong-Min Koo, a professor in the School of Advanced Materials Science and Engineering at South Korea’s Sungkyunkwan University, says. With each attempt to apply the material in a new setting, new behaviors are uncovered.
But that elasticity also means no one research group could possibly explore MXenes’ potential in full. Instead, Gogotsi has become a spokesperson — a salesperson, even — urging collaborators around the world to take part in the pursuit.
The MXene family has expanded to include dozens of different materials, depending on which ingredients are used in the process. The variation is essential to the materials’ tunability — their capacity to be everything to everyone, depending on the task at hand.
Valeria Nicolosi, chair of nanomaterials and advanced microscopy at the School of Chemistry in Trinity College Dublin, began her career studying graphene. After listening to Gogotsi speak at a conference of The Electrochemical Society in Hawaii, she knew she wanted to work with MXenes.
She quickly realized they are easier to process and handle than other two-dimensional nanomaterials, allowing her lab to use MXene solutions — two-dimensional flakes suspended in common solvents like water — to print on plastics, silicon, textiles and fabrics. She and her colleagues can now attempt research that had previously been impossible. Like so many others, they have joined MXene’s worldwide team.
“He has created a network that is a sort of family,” Nicolosi says. “We are all part of this lab. We share objectives and we share this common goal, which is making progress.”
Gogotsi has a “gift of sharing,” Nicolosi says. Where some scientists are protective of their knowledge, he spreads his far and wide. Collaborators describe a leader who is generous, supportive and always available to share in the exploration of new ideas.
“He’s a really amazing captain,” says Maksym Pogorielov, head of the research center and leader of the biomaterials research group at Sumy State University in Ukraine. “I don’t know if he sleeps or not, but usually he replies to any email within five minutes.”
When he first met Gogotsi at a conference five years ago, Pogorielov says, “his presentation absolutely turned my world.” With a background in medicine and biology, Pogorielov’s work focuses on tissue engineering. MXenes’ unique combination of mechanical, thermal, optical and electrical properties have opened new doors in his research, allowing his lab to explore cancer therapy and tissue regeneration while dreaming of other applications to study in time.
Flavia Vitale, assistant professor of neurology and physical medicine and rehabilitation at the University of Pennsylvania, immediately recognized the potential of MXenes to change bioelectronics while watching Gogotsi speak at Penn’s Singh Center for Nanotechnology in 2016.
At the end of the seminar, she ran to him so she didn’t miss the chance to test out the materials as soon as possible. Within weeks, they began working together and soon after became the first to propose MXenes’ use in biomedical devices ranging from invasive brain implants to wearable technology.
Gogotsi was eager to share a bottle of MXenes with Vitale’s students, she says, but more important than that was his willingness to share his expertise and modify MXenes to suit the needs of a particular project.
“He is always available and he’s extremely interested in growing and connecting the community of people working with and on MXenes,” Vitale says.
Gogotsi serves as a mentor to a wide range of students, colleagues and collaborators, many of whom spend time in his lab and then move on to roles in academia, national laboratories and companies where they can expand the pursuit of MXenes’ potential. At a Materials Research Society conference in San Francisco this spring, he saw three former postdoctoral researchers — Gleb Yushin, Junjie Niu and Majid Beidaghi, who have since become tenured faculty at other U.S. institutions, he says.
As the MXene family moves into its second generation, Gogotsi’s lab recently welcomed postdoc Yuan Zhang on a Feodor Lynen Research Fellowship from the Humboldt Foundation. Zhang’s PhD at Germany’s Saarland University was advised by Volker Presser, who co-authored the first MXene paper while on the same fellowship in the same lab more than a decade ago.
“The main product of universities is not research, not papers, not patents,” Gogotsi says. “The main product of universities is people. So the main job for us is teaching and training a new generation of researchers.”
Lucia Gemma Delogu, associate professor in biochemistry at the University of Padua in Italy, calls Gogotsi “a never-ending source of learning.” She was already familiar with MXenes before Vitale, a close friend, introduced her to Gogotsi. After working with other materials for years, including graphene, she found that MXenes have the biocompatible characteristics she needs to investigate the potential for single-cell mass cytometry — an emerging technique that allows the analysis and targeting of individual cells or tissue within the body — to aid in immune regulation. MXenes offer more channels for communication with cells than other materials because of their incredible conductivity.
“I didn’t even know this existed until Lucia found our work,” Gogotsi says. “These things happen all the time. That’s why I’m tirelessly talking to people all over the world. There are smart people everywhere.”
Over the past few years, Gogotsi has sought to bring those people together — at Drexel — by establishing, with Barsoum, the first two international MXene conferences on American soil. (He co-chaired four such conferences that have taken place in China, where roughly 10 times as many MXene-related papers are published each year, he says.)
In 2020, Drexel hosted a virtual conference with more than 2,000 participants and launched a series of weeklong online courses teaching researchers how to make and characterize MXenes. Last year, the in-person second edition of the conference brought to campus hundreds of scientists, journal editors and representatives from industry and funding agencies to discuss the progress thus far and how to push MXenes further. Among the issues raised at the conference was the need to improve MXene synthesis, and in its wake two papers have already been published in Science magazine, the leading U.S. venue for publishing groundbreaking science, in 2023.
MXene sessions and symposia have also begun to appear at other major conferences, Gogotsi says, including those run by the Materials Research Society, American Chemical Society and the Graphene Conferences.
“MXenes are coming of age,” Gogotsi says.
When he’s not traveling to conferences, seminars and lectures, Gogotsi often uses the internet to extend his reach. He recently wrapped a five-week online course for students, scientists and the public at Sumy State University in Ukraine, as part of a broader effort to support his homeland in wartime.
Gogotsi serves as a mentor to a wide range of students, colleagues and collaborators, many of whom spend time in his lab and then move on to roles in academia, national laboratories and companies where they can expand the pursuit of MXenes’ potential.
Gogotsi’s effort to promote MXenes is partly driven by the search for what he calls a “killer application” — the use that will force the materials onto the international stage for industrial manufacture. At the moment, MXenes are largely the province of academic scientists as research on methods to scale up production continues. For now, there is a chicken-and-egg scenario at play: many applications will require mass-scale manufacture of MXenes, but nobody is making them at an industrial scale until a proven application necessitates their manufacture.
Fullerenes, discovered in 1985 and the subject of a Nobel Prize in chemistry in 1996, serve as a cautionary tale in this regard. They are carbon atoms bound in the form of a soccer ball, and their discovery introduced numerous new carbon structures for scientists to study. But although they have found some niche applications, Gogotsi says, they haven’t resulted in the type of broad, practical uses that would make them a household name.
In a relatively short period of time, MXenes appear to be moving in a better direction, but the journey is still ongoing.
“The only way to solve this chicken and egg — this dog trying to catch its tail — is bottom up, for the scientific community to show that it’s worth investing in this,” Nicolosi says. “From there, everything will start.”
Part IV: Limitless Possibility
While the search for a killer application carries on, MXenes have become integral to research efforts in a broad range of important fields, including biomedical devices, electromagnetic interference (EMI) shielding, printable electronics, functional fabrics, water desalination and, of course, energy harvesting and storage — their raison d’être.
“It’s practically limitless at the moment,” says Seon Jun Kim, a senior research scientist at the Korea Institute of Science and Technology who worked in Gogotsi’s lab as a visiting researcher during his PhD in 2014 and returned four years later as a postdoc.
Murata Manufacturing Co., a nearly $40 billion Japanese producer of advanced electronics, was the first industrial partner to buy into MXenes, beginning in 2016 to invest in research at Drexel and license some of the numerous patents Gogotsi and his colleagues have acquired. As the company continues to work toward the production of parts utilizing MXenes’ strengths, others have also expressed interest.
“So much research that goes on in academic labs never sees the light of day — it never crosses that valley of death to get into the commercial marketplace — and really quickly we had a commercial partner who was trying to do just that,” says Aleister Saunders, Drexel’s executive vice provost for research and innovation.
For now, though, MXenes are primarily being explored in academic labs, where so much has already been discovered and so much is still to come. To many in the MXene vortex, EMI shielding — protecting electromagnetic signals from being disrupted or disruptive — offers the clearest path for these materials to leave their mark.
In early 2016, Gogotsi was still focused on using MXenes for energy storage when he listened to Koo, the Korean professor, discuss his research on EMI shielding during a seminar at Drexel. Gogotsi promptly offered him materials to test, and Koo found that MXenes delivered much better results than the composites he had been using. In a matter of months, they had published a paper in Science detailing the unique shielding benefits of MXenes’ layered microstructure — the first time a top journal had published research on EMI shielding. Within about five years, this paper became the most cited publication in the EMI shielding field.
In a world defined by our reliance on electronic and internet-connected devices, there is a growing need for thinner and lighter materials to shield that cacophony of signals.
As a building block, MXenes’ use in EMI shielding could make them ubiquitous. Because of the ease with which they can be printed and processed, combined with their inherent electrical properties, they could contribute to significant leaps forward in the realm of energy storage and harvesting.
In some respects, the medical possibilities of MXenes are even more meaningful. In the early stages of their collaboration, Vitale and Gogotsi found that MXenes could be used to record brain activity as well as state-of-the-art materials like gold and platinum — or in some respects even easier and cheaper, according to Vitale. MXenes could be used to create both wearable and implantable devices with incredible customizability in terms of size, shape and application, she says — tracking brain, heart or muscle activity more simply than is currently done, all without any specialized equipment.
Various projects investigating the possibilities are now being funded by the National Institutes of Health and industry partners, including Vitale’s NIH-backed development of MXene-based brain sensors that would be so easy to use that patients could do so at home or in low-resource settings without specialized neurology centers. Vitale is also developing non-invasive muscle sensors that could help identify tendon or skeletal disorders or better inform rehabilitation.
“I’m very excited about the potential of MXenes to democratize medicine and at the same time personalize health care,” Vitale says.
Pogorielov, who is investigating the use of MXenes in photothermal therapy to treat cancer, suggests that they could be compared with antibiotics because of the diversity of powerful properties they have already displayed in a short period of time. Penicillin was discovered in 1928 and was first used as a treatment in 1941. The first research about MXenes’ potential in biology and medicine, Pogorielov notes, is only six years old.
Elsewhere, MXenes are being studied for their ability to serve as gas sensors, owing to their conductivity and ability to offer gas molecules more surface sites on which to be absorbed. Kim, at KIST, is exploring the development of gas sensors that work at room temperature, which could be implanted in wearable devices or printed onto clothing to detect pollutants in the air, helping to protect people from exposure to toxins.
“The range of applications is what astounds me,” Sharon Walker, dean of Drexel’s College of Engineering, says of MXenes. “It clearly hit a nerve in a positive way. People are identifying it as materials that can solve the issues they have.”
Part V: A Door Opens
Before the discovery of MXenes, Gogotsi had done plenty of work in which he took great pride, including seminal contributions to materials for electrochemical capacitors, which are widely used in industry for high-rate energy harvesting and storage. With his Drexel colleague, Gary Friedman, professor of electrical and computer engineering, he had made advances in the ability to conduct high-resolution analysis of single cells and demonstrated how to use carbon nanotubes as tiny syringe needles to enter the nucleus of a cell. But reproducing the procedures was too difficult and the research community didn’t follow up on his work.
With MXenes, however, he proved with Barsoum, Naguib, his students and post-docs, that materials can be designed atom by atom, combining elements and structures in whatever composition is desired, like a farmer offering chefs around the world the exact ingredients they want to make the dish of their dreams.
Theoretically, he says, there are at least a thousand ways to craft a MXene to its user’s preference, each with its own unique potential.
“The sky is the limit,” Gogotsi says. “We really opened a totally new way of designing and making two-dimensional materials.”
When Naguib reflects on the discovery of MXenes, he describes experiencing “something like euphoria,” he reflects. “…This feeling that you are pushing forward, opening the door for people, and you see behind you thousands of people are entering.”
Those people are the community of MXene researchers who extend across boundaries of space and science in their search to use some of the smallest materials in the world to solve some of its biggest challenges, with Gogotsi their great conductor.
He’s now far removed from the young boy who fell in love with chemistry back in Ukraine, but he retains all of the same energy. Like a poet who writes because he feels compelled, Gogotsi can’t fathom doing anything else but continuing to explore the potential of MXenes and encourage the community he’s built to do the same.
“It’s a true passion for science,” he says. “This is life.
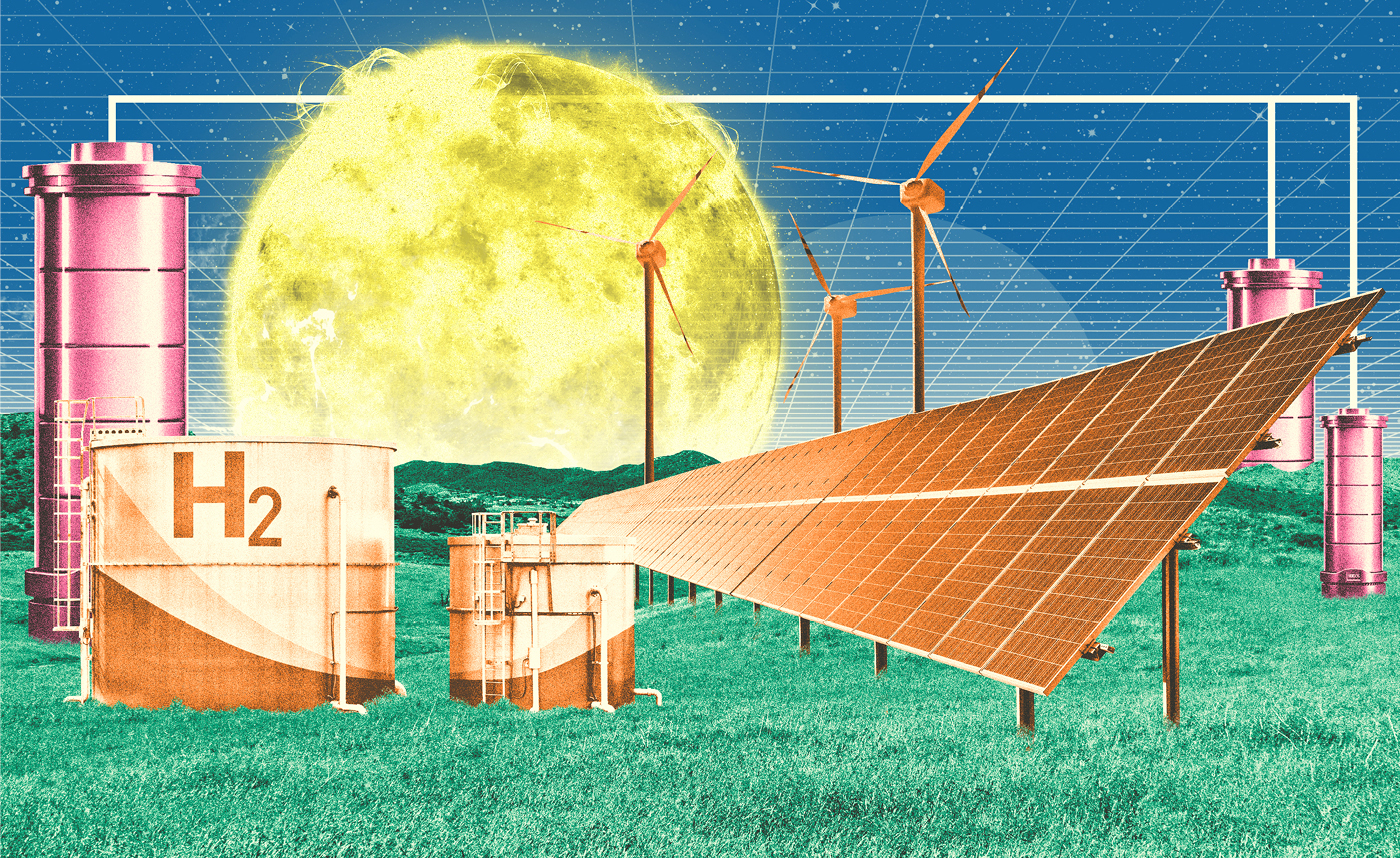
_A Simpler, Greener Wonder Material?
_by Ben Seal
An “idiot-proof” recipe for making a constellation of novel nanomaterials could be the key to sustainable, affordable hydrogen generation.
Michel W. Barsoum is among the trio of Drexel scientists who discovered MXenes in 2011, but these days he’s captivated by something else: a new method to make nanomaterials he’s dubbed HDN that could be the key to sustainable, affordable hydrogen generation.
Like MXenes, nanomaterials made using this approach come with unique and customizable properties. But unlike MXenes, this method requires nothing more than inexpensive, abundant materials, modest heat and time. And it has significant potential for energy, water purification and environmental remediation, says Barsoum, Distinguished Professor in the Department of Materials Science and Engineering in Drexel’s College of Engineering.
For Hussein Badr, who pioneered the HDN technique in Barsoum’s lab, the simplicity of its formula is what makes it so special. Years ago, when he was a research assistant at Cairo University, he was brought short by one of the biggest limitations of MXenes. Like many research labs in countries with limited resources, his had no fume hood — an essential tool for working with hydrofluoric acid, the highly toxic chemical required to synthesize MXenes. The acid is so corrosive it will dissolve a glass container, and its fumes can kill if inhaled in large amounts.
That experience remained with the Egyptian native after he came to Drexel as a doctoral student to study under Barsoum. Barsoum had discovered MXenes with fellow researcher Yury Gogotsi and their doctoral student Michael Naguib a dozen years earlier, and before that, he developed MXenes’ three-dimensional precursor, MAX phases.
“We are losing half of the [world’s researchers] because we’re using one nasty chemical,” Badr says of hydrofluoric acid.
The difficulties of working with the acid were not lost on Barsoum, either, nor on industry partners who were challenging him to find safe, scalable ways to make MXenes.
In 2019, Barsoum and Badr set out to find a method that could eliminate flourine from MXene synthesis.
One year and hundreds of experiments later, Badr stumbled onto a simple and versatile new recipe for making nanomaterials: Place any titanium-containing material, such as titanium boride, and an organic salt, such as tetramethylammonium hydroxide, into a plastic bottle. Heat it to between 50 and 80 degrees Celsius for a few days. Then wash it in alcohol to restore its pH to a workable level.
“I love the idea that I can make a nanomaterial by the kilogram in my kitchen. Those words have never been spoken in a single sentence before.”
—Michel W. Barsoum, Distinguished Professor in the Department of Materials Science and Engineering
Through this “idiot-proof” process, as Barsoum describes it, emerges what he and Badr have termed a “hydroxide-derived nanomaterial,” or HDN. A wide variety of compounds, not only titanium, but also silicon or manganese or iron compounds, can be converted into a plethora of low dimensional materials by simply reacting them in a high pH solution. Barsoum’s focus is on titanium dioxide for now. Additional steps can be incorporated into the process to control the crystallinity, structure and morphology of the final product.
The recipe yields strands of atoms-thin titanium dioxide that Barsoum likens to a familiar food — “the finest spaghetti ever made,” he says. Allowing the alcohol to evaporate results in a balled-up collection of those spaghetti strands. Diluting the material in water, instead, creates a colloidal suspension that, when filtered, results in broader two-dimensional rafts that look something like lasagna noodles composed of individual spaghetti strands, featuring an impressive surface area that holds incredible potential.
Each form offers its own properties, giving researchers the ability to finely tune their creation, depending on its intended purpose.
The food metaphors are apt, given that the entire process requires basic tools available to any home cook. Badr used to tell people it was as easy as boiling an egg. It’s actually even easier, he points out, because less heat is needed.
“It may sound mundane, but I love the idea that I can make a nanomaterial by the kilogram in my kitchen,” Barsoum says. “Those words have never been spoken in a single sentence before.”
After learning how to make HDNs, Barsoum and Badr turned to exploring what they could accomplish with them, using funding from the National Science Foundation, the U.S. Army, Drexel and an industry partner.
Today, nanoscale titanium dioxide — known as P25 — is widely considered the gold standard in some of the applications that are being explored here. Given the easy path to creating the HDN version and its superior properties, including a surface area 20 times greater than commercially sold P25, the entire history of scientific research and thousands of papers on a huge range of topics and applications for the material could be replicated, Badr says. Using the more powerful HDN version could potentially set new standards in many applications.
Perhaps the most meaningful possible application for HDNs is as a stable and inexpensive catalyst in reactions that generate hydrogen. When HDNs in their “spaghetti” form are placed in water and methanol and exposed to sunlight, they create hydrogen at a rate at least one order of magnitude better than than titanium dioxide, Barsoum says.
Considering the Department of Energy’s quest to reduce the cost of clean hydrogen to $1 per kilogram in the next decade, the potential for clean energy is clear.
Imagine a next-generation solar panel system: “In a few years, if this works as well as we think it will, we could be looking at shallow swimming pools in a sunny spot in your yard that generate hydrogen from the sun,” Barsoum says. “Once you have the hydrogen, you can now put it in your tank and run your car on hydrogen. The exhaust is water. This so-called solar hydrogen economy has been and remains, a dream of mankind for a long time.”
HDNs have also proven to be more efficient than standard methods for a process called dye degradation, which can help prevent harmful chemicals from being released into the ecosystem. Because of their high surface area, HDNs can absorb roughly one gram of dye per gram of material — a major step forward. The materials have shown a similar propensity for absorbing uranium from water, which would be a boon to the nuclear industry and in the protection of drinking water systems.
The materials are also biocompatible, opening the door to their use in the biomedical field. They have already been tested and showed promise in cancer therapy, immune cell activation and stem cell differentiation, Badr says.
Just a few short years since the discovery of HDNs, multiple patents have been submitted and papers are rolling in for Barsoum and his colleagues as they continue to explore the materials’ potential and the mechanisms behind the way they work. The group has already found ways to improve their recipe to simplify the process and make it even more affordable.
Meanwhile, they now have more than 100 collaborators around the world — a number that grows as scientists discover the ease of working with the material. Badr says he has spoken with researchers in Benin, Egypt, Saudi Arabia and Morocco, among others, who have now learned to make their own HDNs. With industry partners taking interest as well, HDNs are firmly on the fast track.
“Making a kilogram of any new material, let alone a nanomaterial in your ‘kitchen’ two or three years after discovery is a huge deal,” says Barsoum.
“The fact that within a year or two of our discovery, we’re talking to people about commercialization proves our scalability, affordability, process, ease of making them, stability and technical results,” Badr says. “It also shows that the real world is interested in what we’re doing.”
Charging_While _Driving
Drexel is leading the development of a more forgiving wireless charging system for electric vehicles that could one day enable in-road charging.
Drexel is leading the development of a more forgiving wireless charging system for electric vehicles that could one day enable in-road charging.
Their project, reported in the journal IEEE Transactions on Industrial Electronics, offers a solution to one of the fundamental physical challenges facing all wireless charging technology: misalignment.
Anyone who has accidentally placed their phone on a charging pad a little off kilter — and returned to find it uncharged — can relate. Getting an electric vehicle to line up perfectly over a wireless charger can be an even more challenging task.
The researchers, from Drexel’s College of Engineering and from Shanghai Jiao Tong University, Zhejiang University and Northwestern Polytechnical University in China, designed a more forgiving charging system that is versatile enough to adjust the way it delivers an inductive charge, so that it can accommodate misalignment and various levels of battery charge.
Inductive charging works by using an electromagnetic field to transfer power, similar to the way wind or water might push a turbine to generate electricity. An induction coil receives the electromagnetic “push” from the charger and transforms that vibration into energy that charges a battery.
But, like a misaligned turbine that misses the full force of the wind or water, inductive charging coils that are not properly coupled will not efficiently charge the battery and — with enough misalignment — they may fail to activate the charging process at all.
What the researchers discovered, however, is a method that allows for control over to the frequency of the field, or how quickly or slowly the voltage is being transferred, which can be adjusted for misalignment or how charged the battery is.
“Think about our wireless charging challenge like trying to fill a small glass with water under a tap that’s going at full blast,” explains Fei Lu, an assistant professor at Drexel who is one of the lead researchers. “It can be quite tricky, because if you don’t hold it directly under the tap a lot of water will miss the glass. And once it is nearly full, the force of the stream will splash water out of the glass without it ever being filled to the brim.”
“The key to our system is that it provides more control over the tap — or the charging power, as it were — so it’s easier to direct more of the flow into the glass and slow it down as the glass becomes full,” she says.
They tested the system on both charged and uncharged batteries, under well-aligned and misaligned conditions. The prototype was able to provide a stable power input from 0 to 3.3 kilowatts, which is the standard charging range for plug-in EV charging, with nearly 96% efficiency — on par with commercial wireless chargers and only slightly below that of plug-in chargers.
“Being able to vary the voltage yet maintain the power of the charging system means that this process could be used to efficiently charge both near-dead batteries and those that just need a top-off,” Lu says. “We see this as a critical step in making inductive charging more resilient in real-world conditions.
Behold_the_Battery_Chassis
A battery that doubles as the car frame, combined with its own integrated cooling system, would solve the weight, capacity and durability limits of conventional electric vehicle batteries.
Packing enough energy into a battery to power a car puts a lot of pressure on the storage devices that, for the last century or so, have mainly been tasked with running small appliances and electronics. The stress is getting to them — manifested in malfunctions, diminished performance and even meltdowns.
Researchers are devising ways to improve battery performance by taking some of the literal heat off them.
A team led by Assistant Professor Ahmad Najafi outlined an approach for incorporating a blood-vessel–like cooling network into the packaging of a new generation of solid carbon-fiber–based batteries.
Their method, described in the journal Composites Part B: Engineering, relies on using solid batteries — a thin, carbon fiber-based version of the larger lithium-ion batteries widely used in electric vehicles — that can be cleverly incorporated into the physical structure of the vehicle chassis.
Replacing portions of the car frame with a carbon-fiber composite that functions both as a structural component and as a battery could reduce the overall weight of the vehicle as well as improve its energy-storage capacity. Trimming car weight by just 10% can boost mileage efficiency between charges by an estimated 6–8%.
There’s just one problem: Heat.
“Heat generation will be substantially higher in structural batteries in comparison with standard lithium-ion batteries,” Najafi explains. This is because the conductivity of the polymer electrolyte they use as their medium of electron transit is much smaller than that of the liquid electrolytes used in lithium-ion batteries. So electrons are forced to move more slowly through the polymer and, as a result, they generate more heat as the battery discharges.
Some kind of thermal-management system, therefore, is necessary.
Najafi’s research group has been developing special composite materials for heat management for a number of years. Their work draws on nature’s own cooling method: the vascular system. Modifying a design tool they invented to plot the optimal “microvascular” network, the researchers were able to design cooling composites that would work as part of the structural battery packaging currently being tested by companies like Tesla, Volvo and Volkswagen.
“These composites function something like a radiator in an internal combustion engine vehicle,” Najafi says. “The coolant draws in the heat and pulls it away from the battery composite as it moves through the network of microchannels.”
Sandwiching the structural batteries between layers of cooling microvascular composites can stabilize their temperature and extend their functional time and power range.
Computer models show their system could improve the driving range of a Tesla model S by as much as 23%. But the team notes that the real value of their work is its ability to glean the best combination of battery size and weight — including enough cooling capacity to keep it functioning — for any electric vehicle in production now and any future designs.